Document Type
Article
Publication Date
August 2007
Publication Title
Journal of Geophysical Research: Atmospheres
Volume
112
Issue Number
D16
DOI
10.1029/2006JD008332
Disciplines
Atmospheric Sciences | Climate | Meteorology
Abstract
[1] Simulations from eleven coupled chemistry-climate models (CCMs) employing nearly identical forcings have been used to project the evolution of stratospheric ozone throughout the 21st century. The model-to-model agreement in projected temperature trends is good, and all CCMs predict continued, global mean cooling of the stratosphere over the next 5 decades, increasing from around 0.25 K/decade at 50 hPa to around 1 K/decade at 1 hPa under the Intergovernmental Panel on Climate Change (IPCC) Special Report on Emissions Scenarios (SRES) A1B scenario. In general, the simulated ozone evolution is mainly determined by decreases in halogen concentrations and continued cooling of the global stratosphere due to increases in greenhouse gases (GHGs). Column ozone is projected to increase as stratospheric halogen concentrations return to 1980s levels. Because of ozone increases in the middle and upper stratosphere due to GHG-induced cooling, total ozone averaged over midlatitudes, outside the polar regions, and globally, is projected to increase to 1980 values between 2035 and 2050 and before lower-stratospheric halogen amounts decrease to 1980 values. In the polar regions the CCMs simulate small temperature trends in the first and second half of the 21st century in midwinter. Differences in stratospheric inorganic chlorine (Cly) among the CCMs are key to diagnosing the intermodel differences in simulated ozone recovery, in particular in the Antarctic. It is found that there are substantial quantitative differences in the simulated Cly, with the October mean Antarctic Cly peak value varying from less than 2 ppb to over 3.5 ppb in the CCMs, and the date at which the Cly returns to 1980 values varying from before 2030 to after 2050. There is a similar variation in the timing of recovery of Antarctic springtime column ozone back to 1980 values. As most models underestimate peak Cly near 2000, ozone recovery in the Antarctic could occur even later, between 2060 and 2070. In the Arctic the column ozone increase in spring does not follow halogen decreases as closely as in the Antarctic, reaching 1980 values before Arctic halogen amounts decrease to 1980 values and before the Antarctic. None of the CCMs predict future large decreases in the Arctic column ozone. By 2100, total column ozone is projected to be substantially above 1980 values in all regions except in the tropics.
Recommended Citation
V. Eyring, D. W. Waugh, G. E. Bodeker, Eugene C. Cordero, H. Akiyoshi, J. Austin, S. R. Beagley, B. A. Boville, P. Braesicke, C. Brühl, N. Butchart, M. P. Chipperfield, M. Dameris, R. Deckert, M. Deushi, S. M. Frith, R. R. Garcia, A. Gettelman, M. A. Giorgetta, D. E. Kinnison, E. Mancini, E. Manzini, D. R. Marsh, S. Matthes, T. Nagashima, P. A. Newman, J. E. Nielsen, S. Pawson, G. Pitari, D. A. Plummer, E. Rozanov, M. Schraner, J. F. Scinocca, K. Semeniuk, T. G. Shepherd, K. Shibata, B. Steil, R. S. Stolarski, W. Tian, and M. Yoshiki. "Multimodel Projections of Stratospheric Ozone in the 21st Century" Journal of Geophysical Research: Atmospheres (2007). https://doi.org/10.1029/2006JD008332
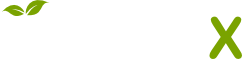
- Citations
- Citation Indexes: 292
- Policy Citations: 13
- Usage
- Downloads: 175
- Abstract Views: 13
- Captures
- Readers: 126
- Mentions
- References: 1
Included in
Atmospheric Sciences Commons, Climate Commons, Meteorology Commons
Comments
This article originally appeared in Journal of Geophysical Research : Atmospheres in Volume 112, Issue D16 and can be found online at this link.